Bridge RNAs: Principles, Applications, And Challenges
By Jie Huang, MD, Ph.D., CSTEAM-Biotech
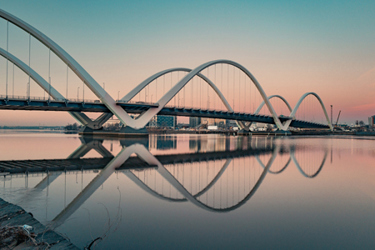
Bridge RNAs are structured noncoding RNAs that facilitate programmable recombination between target and donor DNA, enabling precise genomic rearrangements such as insertions, deletions, and inversions. Their modularity allows for tailored genetic modifications, making bridge RNAs a valuable complement to existing technologies such as CRISPR-Cas and RNA interference. Applications in genome editing, synthetic biology, and genetic diversity research have demonstrated their potential to correct genetic mutations, engineer crops with desirable traits, and produce industrially relevant microorganisms. However, there are also some key challenges that must be addressed. Let’s take a closer look.
Principles Of Bridge RNA
Bridge RNAs exploit natural mechanisms in IS110 insertion sequences, a class of minimal and autonomous mobile genetic elements. These elements can move within the genome, driven by the recombinases they encode, thereby facilitating DNA rearrangements through processes such as homologous recombination and transposition.1 The unique structure of bridge RNAs consists of two internal loops, each encoding a specific nucleotide sequence. These sequences are designed to base pair with both target and donor DNA. The target binding loop of the bridge RNA aligns with the sequence of the DNA site to be modified, while the donor binding loop pairs with the donor DNA, which in this case is typically the IS110 element itself. This dual binding capability enables bridge RNAs to direct precise genetic changes, including insertions, deletions, and inversions of DNA sequences.2
Figure 1: Bridge RNA facilitates DNA recombination by the IS110 recombinase.
One of the significant advantages of bridge RNAs is their modularity. Sequences within the target-binding loop and the donor-binding loop can be reprogrammed independently, enabling highly specific and customizable genome editing. This flexibility means that researchers can tailor bridge RNAs to target a wide range of genomic loci and promote various types of DNA rearrangements.1,2 This customization capability is particularly valuable for genetic engineering, synthetic biology, and therapeutic applications that require precise genetic modifications. In addition, the use of bridge RNAs expands the toolkit of nucleic acid-guided genome editing technologies beyond the well-known CRISPR-Cas and RNA interference systems.
By providing a unified mechanism for the three basic DNA rearrangements (insertion, excision, and inversion), bridge RNAs provide a versatile and powerful approach to genome design and modification. This makes them a promising tool for advancing genetic research and developing new therapeutic strategies to treat genetic diseases.
Applications Of Bridge RNA
Genome Editing
The primary application of bridge RNAs is in genome editing, where they enable precise DNA modifications by guiding sequence-specific recombination. Bridge RNAs do this by containing two internal loops that can base pair with both target and donor DNA. This unique structure enables bridge RNAs to facilitate the insertion, excision, or inversion of specific DNA sequences within the genome.1,2
This level of precision is particularly valuable for correcting genetic mutations that cause disease. For example, in diseases such as cystic fibrosis, where mutations in the CFTR gene lead to defects in the chloride channel, bridge RNAs can be programmed to target and correct these specific mutations. Similarly, in sickle cell anemia, which is caused by a single nucleotide mutation in the HBB gene, bridge RNAs can be used to correct the defective gene, potentially providing a therapeutic solution.3,4
Bridge RNAs offer several advantages over traditional genome editing tools. Their modularity allows for independent reprogramming of the target-binding loop and the donor-binding loop, providing greater flexibility and specificity for genetic modification, complementing technologies such as CRISPR-Cas9, which also have high precision but rely on creating double-strand breaks in the DNA.5
The ability to precisely edit the genome using bridge RNA has great potential for developing therapies for genetic diseases. By correcting the underlying genetic defect, these therapies could address the root cause of the disease, providing more effective and durable treatments. In addition, the use of bridge RNA could facilitate the production of transgenic organisms with desirable traits, such as enhanced disease resistance or improved metabolic function, which is critical to advances in agricultural biotechnology and synthetic biology.
Synthetic Biology
In synthetic biology, bridge RNA plays an important role in engineering organisms with desirable traits by inserting specific gene sequences. This capability helps breed crops that are more resistant to pests and diseases, have higher nutritional content, or are better adapted to environmental stress.6 For example, inserting genes that confer drought resistance into crop genomes could significantly increase agricultural productivity and ensure food security in water-scarce regions.
Bridge RNA enables precise genome modifications, which are essential for optimizing traits such as insect resistance. For example, by introducing genes that produce natural insecticides or enhance plant immune responses, crops can be more effectively protected against pest attacks, thereby reducing the need for chemical pesticides and promoting sustainable agriculture. In addition, improving nutritional content by inserting genes responsible for increased vitamin or protein production can address malnutrition in vulnerable populations.7
The precision and modularity of bridge RNA make it a powerful tool in synthetic biology, driving advances in agricultural systems and ultimately benefiting the environment and human health.
Genetic Diversity And Evolution Studies
Bridge RNAs have also facilitated studies of genetic diversity and the mechanisms of DNA repair and recombination. By enabling precise genome rearrangements, they allow researchers to study how genetic variation arises and contributes to evolution.1,2 This understanding could shed light on the natural processes that drive genetic diversity, helping scientists better understand the evolutionary dynamics of different species.
Complementary Tools To Existing Technologies
Bridge RNAs provide complementary tools to existing genome editing technologies such as the CRISPR-Cas system and RNA interference. While CRISPR-Cas9 is widely used for its ability to introduce double-strand breaks at specific genomic locations, bridge RNAs offer an alternative approach to targeted genome modification without such breaks. This can reduce the risk of unintended off-target effects and enhance the specificity of genetic modifications. Furthermore, the modularity of bridge RNAs allows for independent reprogramming of its target-binding and donor-binding loops, providing greater flexibility and control over the editing process.1,2
Advances In Biotechnology
In biotechnology, bridge RNA facilitates the creation of genetically modified microorganisms for industrial applications. Engineered bacteria with optimized metabolic pathways can be developed to more efficiently produce biofuels, pharmaceuticals, or other valuable biochemicals. By precisely controlling the genetic makeup of these microorganisms, bridge RNA can significantly enhance metabolic engineering efforts, thereby driving major advances in industrial biotechnology.1,2 This precise genome editing capability can optimize production processes, increase yields, and reduce costs, thereby revolutionizing the production of key industrial compounds and enhancing sustainability across industries.
Key Challenges To Overcome With Bridge RNAs
Despite their great potential, bridge RNAs face several challenges that must be addressed to fully realize their applications in genome editing. The main issue is to ensure the specificity and efficiency of the recombination process to avoid off-target effects. Off-target recombination can lead to unintended genetic alterations, which can have harmful consequences, including activation of oncogenes or disruption of essential genes.8 Improving the precision of bridge RNAs is essential to minimize these risks.
Delivering bridge RNAs into cells is another major challenge. Effective delivery methods must ensure that the bridge RNA reaches its target cells and maintains its functionality in the cellular environment. Current delivery technologies, such as viral vectors, lipid nanoparticles, and electroporation, all have their limitations and can affect the stability and efficiency of bridge RNAs. Optimizing these delivery methods is essential for the successful application of bridge RNAs in vivo. In addition, extensive research is needed to understand the full biological impact and potential side effects of bridge RNA-mediated recombination.1,2 This includes studying the long-term effects of genome editing and possible unintended genetic consequences.
Ethical and regulatory issues also pose significant challenges. The use of advanced genetic engineering technologies such as bridge RNAs must be strictly regulated to ensure safety and ethical compliance. This involves developing strong regulatory frameworks to address the potential risks and ethical issues associated with genome editing, particularly in human applications.1,2
Meeting these challenges will require concerted efforts in research, development, and regulatory oversight to fully exploit the potential of bridge RNA while ensuring its safe and ethical use.
Conclusion
Bridge RNAs represent a transformative advance in genome editing, enabling precise and programmable recombination between target and donor DNA sequences. Bridging RNAs exploit the natural mechanism of IS110 insertion sequences to facilitate the necessary genomic rearrangements (insertions, deletions, and inversions) through their structured noncoding RNA loops that specifically bind to both target and donor DNA. This modularity and reprogrammability enhance their versatility, expanding the genetic engineering toolkit beyond CRISPR-Cas systems and RNA interference. Bridging RNAs have tremendous potential for application in genetic research, synthetic biology, and therapeutic interventions, enabling precise genetic modification and the development of organisms with desirable traits. However, challenges such as ensuring specificity, efficient delivery, and addressing ethical and regulatory issues must be overcome. Continued research and development are essential to fully exploit the potential of bridge RNAs and integrate them safely and effectively into practical applications.
References
- Matthew Durrant et al. (2024), Bridge RNAs direct programmable recombination of target and donor DNA. Nature (https://doi.org/10.1038/s41586-024-07552-4)
- Masahiro Hiraizumi et al. (2024), Structural mechanism of bridge RNA-guided recombination. Nature (https://doi.org/10.1038/s41586-024-07570-2)
- Karine Deletang and Magali Taulan-Cadars (2022), Splicing mutations in the CFTR gene as therapeutic targets. Gene Therapy (https://doi.org/10.1038/s41434-022-00347-0)
- Tania Carlice-dos-Reis et al. (2017), Investigation of mutations in the HBB gene using the 1,000 genomes database. PLoS One (10.1371/journal.pone.0174637)
- Gabriel Longo et al. (2024), Linking CRISPR–Cas9 double-strand break profiles to gene editing precision with BreakTag. Nature Biotechnology (https://doi.org/10.1038/s41587-024-02238-8)
- Blaine Pfeifer et al. (2023), Harnessing synthetic biology for advancing RNA therapeutics and vaccine design. NPJ System Biology and Applications (https://doi.org/10.1038/s41540-023-00323-3)
- Miguel Altieri et al. (2024), Towards an agroecological approach to crop health: reducing pest incidence through synergies between plant diversity and soil microbial ecology. NPJ Sustainable Agriculture (https://doi.org/10.1038/s44264-024-00016-2)
- Jennifer Doudna (2020), The promise and challenge of therapeutic genome editing. Nature (https://doi.org/10.1038/s41586-020-1978-5)
About The Author:
Jack (Jie) Huang, MD, Ph.D., is the chief scientist and founder/CEO at CSTEAM Biotechnology in Ohio, USA. He is also recognized as a medical science writer, an inventor, and a STEM educator. Huang completed his MD/Ph.D. at Shiga University of Medical Science (SUMS) in Japan in 2000. He then pursued postdoctoral training in immunology at the University of Tokyo, followed by a role as research assistant professor at Tohoku University. In the U.S., he served as a postdoctoral fellow at Oregon Health & Science University and as an oncologist and senior research scientist at Ohio State University Nationwide Children’s Hospital, focusing on oncology research until 2018, when he launched his company specializing in biological models and biochips. Additionally, Huang is vice president of the American Botanical Drug Association and director of the Stem Cell Engineering and Technology Research Center at the Industry Technology Research Institute of Chongqing University. His research interests, which span genetic engineering biological models, gene-edited stem cells, immune cell drugs, and biochip technology, have led to numerous patents.